BILL WICKNER LAB at Dartmouth
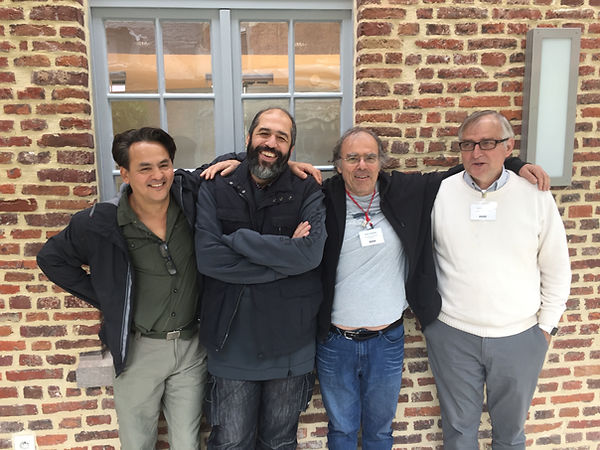
Franck, Tassos, Andreas, Ross
PROTEIN TRANSLOCATION DISCOVERIES OF THE LAB
Post-translational Translocation -Mandel, Ito, Date, Watts, Silver, Zimmermann, Ohno- Iwashita, Kuhn, Kreil, Bacallao
Translocation information in mature as well as pre-sequence-Date, Kuhn, Dalbey, Kreil, von Heijne, Lill
Protein translocation requiring ATP and -Date, Zwizinski, Goodman, Geller, Schiebel, Driessen, Hartl, Arkowitz, Bassilana
Leader peptidase purification, gene, sequence, topology-Zwizinski, Date, Dalbey, Wolfe, Silver, Watts, von Heijne
SecA is an ATPase-Cunningham, Lill
SecA ATPase is activated by lipid, SecYEG, leader & mature sequences -Cunningham, Lill, Hendrick, Bassilana, Arkowitz
SecYEG is the integral domain of preprotein translocase -Brundage, Driessen, Schiebel, Hendrick, Fimmel
Chaperones allow post-translational translocation - Crooke, Brundage, Lecker, Lill, Guthrie
High affinity binding of SecB to SecA, and SecA to SecYEG -Hartl, Lecker, Schiebel
SecA couples ATP binding to insertion into SecYEG, translocating a preprotein segment -Economou, Price, Duong, Eichler
Preprotein passes from SecA to SecYEG, shielded from lipid- Joly
translocates preprotein when not engaged with SecA -Schiebel, Driessen, Hartl
Hydrophobicity governs lateral release from SecYEG-Duong
SecYE is the translocase core, aided by SecG or, with , SecDFYajC -Arkowitz, Duong
Preprotein translocase holoenzyme is SecAYEGDFYajC -Duong
And later, by lab alumni
Leader peptidase structure and novel catalytic dyad -Dalbey
The leader (signal) sequence allosterically activates translocase -Economou
YidC strongly enhances SecA-independent translocation -Dalbey, Kuhn
Our lab worked on protein translocation for decades before taking up vacuole fusion. Rather than review here the development of the whole field of protein translocation or even bacterial protein export, I present some whimsical recollections of our lab's contributions. My own path to translocation began in 1968, when I started work in Eugene Kennedy's lab, shamelessly minimizing my medical studies to indulge a growing passion for biochemistry while avoiding Vietnam. Active membrane enzymes hadn't been purified yet, so I was assigned the purification of phosphatidylserine decarboxylase. The source was a 100 lb block of frozen E. coli paste which arrived at 2 a.m. at Logan Airfreight from the Grain Processing Corporation of Muscatine, Iowa. It took years to figure out how to solubilize and purify this enzyme with "Cutscum" (a floor wax detergent, later named Triton X100). Phosphatidylserine decarboxylase was purified 3,500-fold to near homogeneity with a 5% yield, in an era before cloning and overexpression (1). The role of detergent was unclear at that time, and enzymology experts advised me to remove the detergent by ether extraction once the enzyme had been liberated from membranes. Invariably, the protein crashed out of solution when I did so, and I was thus driven to keep the detergent present throughout. This was well before the fluid mosaic model, which came along in 1972 (2). In this same era, the components and mechanisms of protein synthesis were being resolved and reconstituted, and none of them involved membranes. How did a membrane-bound protein get synthesized without the kind of aggregation I saw when I tried to remove Triton during phosphatidylserine decarboxylase purification?
This question gnawed at me during my postdoc, so when I began working independently, I hypothesized that cells had an undiscovered detergent which they used to keep proteins in solution until the protein could leave the detergent and slip into a membrane. To purify this mythical detergent, I performed successive and alternate extractions of E. coli with diethylether, then water, then ether, then water. This yielded a pure compound... which turned out to be the antifoam from the fermenter! A pretty inauspicious start for an eager young scientist, especially as we were expecting our first child soon. So I scrubbed my bench, which I do in times of stress, and sought advice about what membrane protein I should study from the perspective of its biosynthesis. Doug Brutlag suggested that I consider the coat protein of coliphage M13, a virus-encoded plasma membrane protein that assembles onto extruding viral DNA to form virions. Though only 50 amino acyl residues long, M13 coat protein was readily purified in gram quantities from virus, and its sequence had been determined by classical peptide chemistry and sequential manual Edman degradation, a complete rarity for a membrane protein in the era before DNA sequencing. The first 20 residues of coat protein are polar and acidic, its next 20 residues apolar, and its C-terminal 10 residues are basic. M13 coat protein is initially made with an extra 23 N-terminal amino-acyl residues, termed a leader or signal sequence. Since the signal hypothesis emphasized exclusively co-translational translocation, I preferred the neutral term "leader".
For a decade we focused on M13 coat protein biogenesis (Figure 1), first when my lab was just me and then later as others joined in. Pulse-chase studies with M13-infected E. coli revealed salient features of its biogenesis: 1. It's made as procoat, a 73 amino acid precursor form, but then processed post-translationally to its mature size of 50 residues (3, 4). This was heresy, as exported proteins were believed to only co-translationally translocate. Were we seeing post-translational translocation, or just slow proteolytic processing?
Procoat membrane assembly required more than just a somehow unique leader (signal) sequence (5), and even the C-terminal residues which never translocate into the bilayer are required for membrane assembly (6). 2. When pulse-labeled cells were spheroplasted and treated with external protease, procoat was inaccessible (Figure 2). After its cleavage to coat protein, its N-terminal 20 residues were exposed to the periplasm, and it spanned the plasma membrane (7). 3. The plasma membrane electrochemical potential was required for procoat translocation, and for translocation of virtually all proteins into the periplasm (Figure 3), allowing a clear separation of its synthesis from its membrane transit (7- 9). 4. The SEC gene encoded proteins, discovered by Beckwith and colleagues in this era, were not needed for procoat membrane assembly (10). 5. Procoat membrane insertion could be reconstituted with ever-increasing clarity into liposomes, liposomes loaded with lumenal proteases (Figure 4), or liposomes with leader peptidase (11, 12).
Though procoat membrane-insertion can occur without catalysis, later studies by Andreas Kuhn and Ross Dalbey in their own labs (13) were to show that there is a catalyst, YidC, which improves the reaction and was shown to be important for all plasma membrane proteins. Arthur Kornberg was fond of noting that nature never failed to evolve a catalyst, as even the facile reaction of bicarbonate plus a proton to water plus CO2 is greatly accelerated by carbonic anhydrase.
The maturation of procoat to coat provided us an assay for the purification of leader (signal) peptidase (14). Assay of extracts of cells in the Clark & Carbon collection, each with a multicopy plasmid bearing a small patch of the E. coli genome, led to the identification and mapping of the LEP gene for the enzyme and creation of an overexpression strain (15), allowing purification of milligrams of leader peptidase (16). The continuing work on M13 procoat was now joined by a multi-pronged study of leader peptidase: 1. Its substrate specificity, 2. Its membrane topology, 3. Its own biogenesis. Leader peptidase has 2 apolar membrane spanning domains, both near its N-terminus. The very N-terminus is exposed to the periplasm, then it spans the membrane, exposes a short polar region (which separates the two apolar domains) to the cytoplasm, then spans the membrane again, and its large, polar C-terminal domain is anchored to the periplasmic surface of the plasma membrane. The membrane assembly of leader peptidase requires each of the Sec proteins (17), a clear contrast to procoat, and this assembly could again occur post-translationally. Nevertheless, there was no cleaved leader sequence, suggesting that targeting information might reside in the mature protein domain, a concept that was unequivocally proven in following years (18, 19).
To complement studies of these two integral plasma membrane proteins, we began studies of the biogenesis of Outer Membrane Protein A, or OmpA. LIke procoat, it is also synthesized in a precursor form, proOmpA, with a typical N-terminal leader sequence. ProOmpA became the workhorse substrate for studies of Sec-dependent protein translocation, and remains so to this day. ProOmpA export requires both ATP and the membrane electrochemical potential (20, 21; Figure 5). For each of these proteins-- M13 procoat, Lep, and proOmpA-- our pattern of study was similar, encompassing studies of the kinetics, topology, energy requirements, and proteolytic processing in vivo, purification of the substrate preprotein, reconstitution of the translocation of in vitro synthesized radioactive precursor or purified preprotein into sealed, inverted inner membrane vesicles, and culminating with reconstitution of the translocation with all-purified components (11, 22, 23).
To reconstitute Sec-dependent translocation, we began by purifying active SecA (27), then finding conditions where membranes could be detergent-solubilized and functionally reconstituted, albeit without fractionation (24). Arnold Driessen then introduced the lab to earlier findings that transport systems were best reconstituted with reduced water concentrations after solubilization with detergent/lipid mixed micelles instead of detergent alone (25). This was successfully applied to E. coli inner membrane vesicles (24, 26), and fractionation of the mixed micellar extract yielded a trimeric complex (23) of SecY, SecE, and a third protein which we intially gave the vanilla-flavored name "band 1" but was later termed SecG (Figure 6A-C). SecYEG, after reconstitution into proteoliposomes with bacteriorhodopsin and given SecA (27) and ATP, translocated proOmpA in a light and ATP-dependent reaction (Figure 6D, E; ref. 23). SecAYEG forms the core of preprotein translocase of E. coli. Later studies showed that SecA can function with SecY alone for a basal translocase activity, strongly stimulated by SecE. SecG or SecDFYajC further enhance translocase function, and gentle extraction in digitonin revealed that SecYEGDFYajC (Figure 7A) are together as part of a preprotein translocase holoenzyme (28). More recent studies by Collinson's group (29) support such a SecAYEGDFYajCYidC holoenzyme and reveal its surprising lipidic core. Nevertheless, the purification of functional SecA and SecYEG was a milestone achievement for us, and led to a decade of productive studies of how translocase works.
Preproteins are stabilized by chaperones (30, 31). One export-specific chaperone, SecB, has a direct affinity for SecA (30). SecA couples ATP binding energy to driving translocation (27, 32, 33). One night in 1988, at 3:00 in the morning, the Munich household of Walter and Monika Neupert was awoken by a phone call for me from Kyle Cunningham and Roland Lill in California. They had discovered that the ATPase activity of SecA was activated 100-fold by its simultaneous engagement with acidic membrane lipids, SecYEG, and the leader and mature domains of a preprotein such as proOmpA (Figure 8), and knew I'd want to hear this right away (18)! The binding of ATP to SecA drives approximately 20 amino acyl residues of preprotein forward through SecYEG (32, 34). SecA achieves this by undergoing a large conformational change in which a major domain becomes protease-inaccessible from the cytoplasmic membrane surface and may even become accessible to small molecules from the periplasm (35-37). The chain is released upon ATP hydrolysis (Figure 9), and could backslide except for the forward translocation energy from the membrane electrochemical potential (32, 34). Studies with proOmpA-bound photoactivable nitrene crosslinkers showed that segments of preprotein are initially bound to SecA, then pass to SecY while remaining shielded from lipid before completing their membrane transit (38). Apolar segments of the preprotein cause a pause in translocation and mediate lateral release into the lipid bilayer (39).
This work, from 1974 to 2002, was embedded in an exciting, growing field which Randy and I reviewed at the end of this era (40), but the foregoing is simply a retrospective of our lab's contributions. Beckwith, Silhavy, Oliver, Kumamoto, and colleagues identified the SEC/PRL genes for the proteins which we and Shoji Mizushima came to biochemically. Our work was immeasurably enriched and accelerated by their generosity with strains and insights. Protein import into mitochondria was brilliantly studied by Neupert, soon joined by Schatz. MItochndrial protein import has distinct pre-sequence motifs, but a shared need for membrane potential. It also needs ATP, but mitochondria use ATP to help pull preprotein chains rather than the "push" mechanism of SecA. Direct, often heated, comparisons were drawn between bacterial translocation and signal recognition particle-dependent, obligately cotranslational translocation into the ER. Tom Rapoport's group showed that the membrane-embedded domain of canine ER translocase was homologous to the trimeric SecYEG we had discovered, yet there is no ER homolog of SecA. Of course our understanding of preprotein translocase (SecAYEG) has been taken to another level by structural studies from Tom's group, first of SecYEG (41), then of SecAYEG (42), and most recently of SecAYEG with a transiting membrane chain (43), and by Ian Collinson's group's exciting structure of the preprotein translocase holoenzyme (29).
My teacher, Gene Kennedy, said that two problems should be addressed in a scientific life, with a couple of decades devoted to each. It's not that one has "solved" the first problem after only 20 years, but that you've done what you know how to do, and a fresh challenge is good. During "Midlife Crisis", an academic might change University, change spouse, change scientific problem, or buy a red Porsche. No Porsche for me, and Hali and I remain in love, but I did change universities and undertook another problem, vacuole inheritance and fusion. However, my passion for translocation remained during this transition and after, and our earlier work attracted talented postdocs who, alongside a growing lab interest in vacuole fusion, continued studies of the translocase mechanism.
References
1. Dowhan, W., Wickner, W. and Kennedy, E.P. (1974). Purification and properties of phosphatidylserine decarboxylase from Escherichia coli. J. Biol. Chem. 249, 3079-3084.
2. Singer, S.J. and Nicholson, G.L. (1972) The fluid mosaic model of the structure of cell membranes. Science 174, 720-731.
3. Ito, K., Mandel, G. and Wickner, W. (1979). Soluble precursor of an integral membrane protein: Synthesis of procoat protein in Escherichia coli infected with bacteriophage M13. Proc. Natl. Acad. Sci. U.S.A. 76, 1199-1203.
4. Ito, K., Date, T. and Wickner, W. (1980). Synthesis, assembly into the cytoplasmic membrane, and proteolytic processing of the precursor of coliphage M13 coat protein. J. Biol. Chem. 255, 2123-2130.
5. Kuhn, A., Kreil, G. and Wickner, W. (1987). Recombinant forms of M13 procoat with an OmpA leader sequence or a large carboxy-terminal extension retain their independence of secY function. EMBO J. 6, 501-505.
6. Kuhn, A., Wickner, W. and Kreil, G. (1986). The cytoplasmic carboxy terminus of M13 procoat is required for the membrane insertion of its central domain. Nature 322, 335-339.
7. Date, T. and Wickner, W. (1980) Procoat, the precursor of M13 coat protein, inserts post-translationally into the membrane of cells infected by wild-type virus. J. Virol. 37, 1087-1089.
8. Date, T., Zwizinski, C., Ludmerer, S. and Wickner, W. (1980). Mechanisms of membrane assembly: The effects of energy poisons on the conversion of soluble M13 procoat to membrane-bound coat protein. Proc. Natl. Acad. Sci. U.S.A. 77, 827-831.
9. Date, T., Goodman, J.M. and Wickner, W. (1980). Procoat, the precursor of M13 coat protein, requires an electrochemical potential for membrane insertion. Proc. Natl. Acad. Sci. U.S.A. 77, 4669-4673.
10. Wolfe, P., Rice, M. and Wickner, W. (1984). Effects of the two sec genes on protein assembly into the plasma membrane of Escherichia coli. J. Biol. Chem. 260, 1836-1841.
11. Watts, C., Silver, P. and Wickner, W. (1981). Membrane assembly from purified components. II. Proteolytic processing and insertion of M13 procoat into liposomes reconstituted with purified leader peptidase. Cell 25, 347-353.
12. Geller, B.L. and Wickner, W. (1985). M13 procoat inserts into liposomes in the absence of other membrane proteins. J. Biol. Chem. 260, 13281-13285.
13 Serek, J, BVauer-Manz, G, Struhalla, G, van den Berg, L, Kiefer, D, Dalbey, R, and Kuhn, A (2004) Escherichia coli YidC is a membrane invertase for Sec-independent proteins. EMBO J. 23, 294-301.
14. Zwizinski, C. and Wickner, W. (1980). Isolation and characterization of leader (signal) peptidase from Escherichia coli. J. Biol. Chem. 255, 7973-7977.
15. Date, T. and Wickner, W. (1981). Isolation of the E. coli leader peptidase gene and effects of its overproduction in vivo. Proc. Natl. Acad. Sci. U.S.A. 78, 6106-6110.
16. Wolfe, P.B., Silver, P. and Wickner, W. (1982). Membrane assembly from purified components. III. The isolation and homogeneous leader peptidase from a strain of Escherichia coli which overproduces the enzyme. J. Biol. Chem. 257, 7898-7902.
17. Wolfe, P.B. and Wickner, W. (1984). Bacterial leader peptidase, a membrane protein without a leader peptide, uses the same export pathway as pre-secretory proteins. Cell 36, 1067-1072.
18. Lill, R., Dowhan, W., and Wickner, W. (1990). The ATPase activity of SecA is regulated by acidic phospholipids, SecY, and the leader and mature domains of precursor proteins. Cell, 60, 271-281.
19. Gouridis, G, Karamanou, S, Gelis, I, Kalodimos, CG, and Economou, A (2009) Signal peptides are allosteric activators of the protein translocase. Nature 462, 363-368.
20. Zimmermann, R. and Wickner, W. (1983). Energetics and intermediates of the assembly of protein OmpA into the outer membrane of Escherichia coli. J. Biol. Chem. 258, 3920-3925.
21. Geller, B.L., Movva, N.R. and Wickner, W. (1986). Both ATP and the electrochemical potential are required for optimal assembly of pro-OmpA into Escherichia coli inner membrane vesicles. Proc. Natl. Acad. Sci. U.S.A. 83, 4219-4222.
22. Ohno-Iwashita, Y. and Wickner, W. (1983). Reconstitution of rapid and asymmetric assembly of M13 procoat protein into liposomes which have bacterial leader peptidase. J. Biol. Chem. 258, 1895-1900.
23. Brundage, L., Hendrick, J.P., Schiebel, E., Driessen, A.J.M., and Wickner, W. (1990). The purified E. coli integral membrane protein SecY/E is sufficient for reconstitution of SecA-dependent precursor protein translocation. Cell 62, 649-657.
24. Cunningham, K. and Wickner, W. (1989). Detergent disruption of bacterial inner membranes and recovery of protein translocation activity. Proc. Natl. Acad. Sci. USA, 86, 8673-8677.
25 Ambudkar, S.V. and Maloney, P.C. (1986) Bacterial anion exchange. Use of osmolytes during solubilization and reconstitution of phosphate-linked antiport from Streptococcus lactis. J. Biol. Chem. 261, 10079-10086; Newman, M.J. and Wilson, T.H. (1980) Solubilization and reconstitution of the lactose transport system from Eschericia coli. J. Biol. Chem. 255, 10583-10586.
26. Driessen, A.J.M. and Wickner, W. (1990). Solubilization and functional reconstitution of the protein translocation enzymes of Escherichia coli. Proc. Natl. Acad. Sci. USA, 87, 3107-3111.
27. Cunningham, K., Lill, R., Crooke, E., Rice, M., Moore, K., Wickner, W., and Oliver, D. (1989). Isolation of SecA protein, a peripheral protein of the E. coli plasma membrane that is essential for the functional binding and translocation of proOmpA. EMBO J., 8, 955-959.
28. Duong, F. and Wickner, W. (1997). Distinct catalytic roles of the SecYE, SecG, and SecDFYajC subunits of preprotein translocase holoenzyme. EMBO J. 16, 2756-2768.
29. Martin, R., Larsen, A.H., Corey, R.A., Midtgaard, S.R., Frielinghaus, H., Schaffitzel, C., Arleth, L., and Collinson, I (2019) Structure and dynamics of the central lipid pool and proteins of the bacterial holo-translocon. Biophys. J. 116, 1931-1940; Komar, J., Alvira, S., ..., and Collinson, I. (2016) Membrane protein insertion and assembly by the bacterial holo-translocon SecYEG-SecDF-YajC-YidC. Biochem J. 473, 3341-3354; Schulze, R.J., Komar J., ..., and Collinson, I (2014) Membrane protein insertion and proton-motive-force-dependent secretion through the bacterial holo-translocon SecYEG-SecDF-YajC-YidC. Proc. Natl. Acad. Sci. USA 111, 4844-4849.
30. Hartl, F.-U., Lecker, S., Schiebel, E., Hendrick, J.P., and Wickner, W. (1990). The binding of SecB to SecA to SecY/E mediates preprotein targeting to the E. coli membrane. Cell 63, 269-279.
31. Lecker, S., Lill, R., Ziegelhoffer, T., Bassford, P.J. Jr., Kumamoto, C.A., and Wickner, W. (1989). Three pure chaperone proteins of Escherichia coli, SecB, Trigger Factor, and GroEL, form Soluble Complexes with Precursor Proteins in vitro. EMBO J., 8, 2703-2709.
32. Schiebel, E., Driessen, A.J.M., Hartl, F.-U., and Wickner, W. (1991). and ATP function at different steps of the catalytic cycle of preprotein translocase. Cell 64, 927-939.
33. Lill, R., Cunningham, K., Brundage, L., Ito, K., Oliver, D., and Wickner, W. (1989). The SecA protein hydrolyzes ATP and is an essential component of the protein translocation ATPase of E. coli. EMBO J., 8, 961-966.
34. Morita, K., Tokuda, H., and Nishiyama, K-i (2012) Multiple SecA molecules drive protein translocation across a single translocon with SecG inversion. J. Biol. Chem. 287, 455-464.
35. Economou, A. and Wickner, W. (1994). SecA promotes preprotein translocation by undergoing ATP-driven cycles of membrane insertion and deinsertion. Cell 78, 835-843.
36. Economou, A., Pogliano, J.A., Beckwith, J., Oliver, D., and Wickner, W. (1995). SecA membrane cycling at SecYEG is driven by distinct ATP binding and hydrolysis events and is regulated by SecD and SecF. Cell 83, 1171-1181.
37. Eichler, J. and Wickner, W. (1998) The SecA subunit of Escherichia coli preprotein translocase is exposed to the periplasm. J. Bacteriol. 180, 5776-5997.
38. Joly, J.C. and Wickner, W. (1993). The SecA and SecY subunits of translocase are the nearest neighbors of a translocating preprotein, shielding it from phospholipids. EMBO J. 12, 255-263.
39. Duong, F. and Wickner, W. (1998) Sec-dependent membrane protein biogenesis: SecYEG, preprotein hydrophobicity and translocation kinetics control the stop-transfer function. EMBO J. 17, 696-705.
40. Wickner, W. and Schekman, R. (2005) Protein translocation across biological membranes. Science 310, 1452-1456.
41. van den Berg, B., Clemons, W.M. Jr., Collinson, I., Modis, Y., Hartmann, E., Harrison, S.C., and Rapoport, T.A. (2003) X-ray structure of a protein-conducting channel. Nature 427, 36-44.
42. Zimmer, J., Nam, Y., and Rapoport, T.A. (2008) Structure of a complex of the ATPase SecA and the protein-translocation channel. Nature 455, 936-943.
43. Ma, C., Wu, X., Sun, D., Park, E., Catipovic, M.A., Rapoport, T.A., Gao, N., and Li, L. (2019) Structure of the substrate-engaged SecA-SecY protein translocation machine. Nature Comm. doi.org/10.1038/s4167-019-10918-2
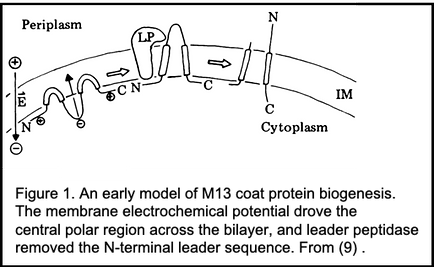
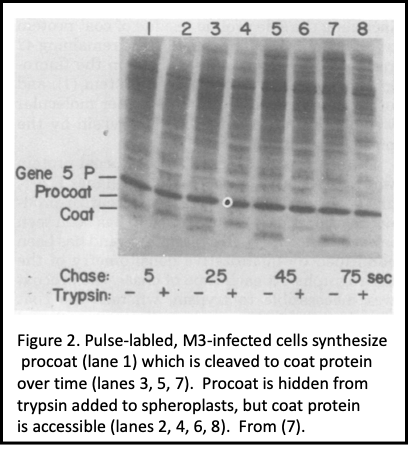
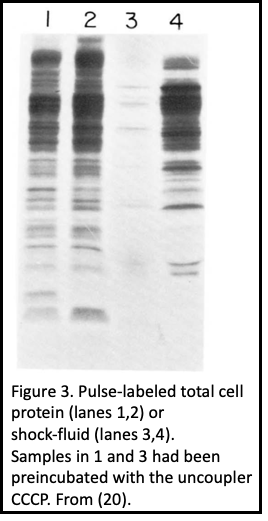

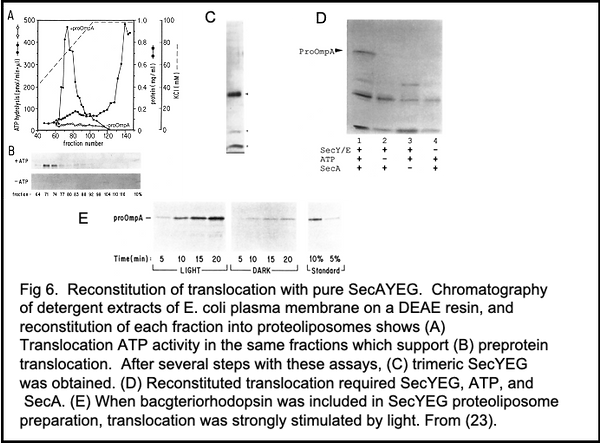

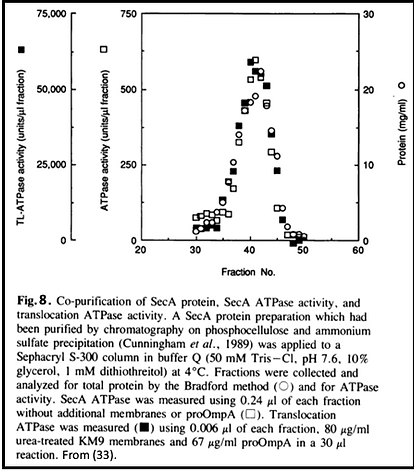





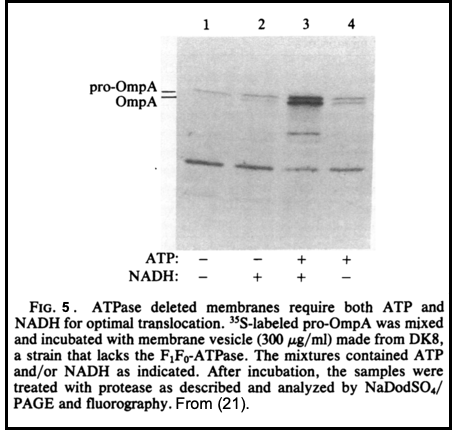